Digestive System On a Chip
The vasculature is the main structure of the circulatory system through which nutrients, oxygen and biological secretions are transported throughout the body. This large network has specific structures regarding their functions. Among all the vital ones, our vasculature carries blood from the largest arteries towards the tiniest capillaries. The functional disorders of the vascular system are closely linked to critical disease processes such as cancer metastasis, tumor angiogenesis or even atherosclerosis. Thus, there has been long-standing interest in the recapitulation of the structure of the microvasculature to gain more insight into the mechanisms of vascular diseases.
In this short review, we outline how engineered microfluidic devices have shifted the paradigm of relevant physiological vasculature. We also discuss some articles demonstrating recent cutting-edge methods to develop vasculature on a chip.
Human vasculature: a complex and dynamic 3D structure
Before trying to engineer vasculature on a microfluidic chip, it is important to understand the natural process of vascular formation and remodeling in vivo. Two different mechanisms can be distinguished during the formation of natural vessels (Figure 1). The first, vasculogenesis, occurs during the embryonic stage, and differentiates angioblasts into endothelial cells to form the blood circulatory system. The second, angiogenesis, follows the vasculogenesis, either to expands the circulatory system as the body grows or during wound healing processes, and sprouts new blood vessels from pre-existing ones [1].
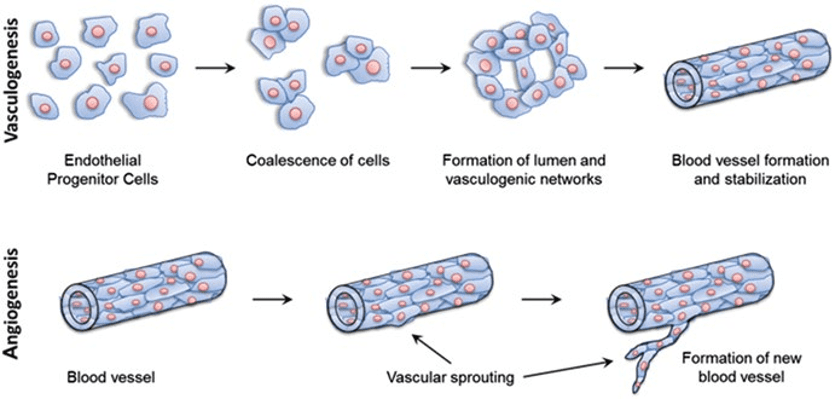
Figure 1 – Blood vessel formation via vasculogenesis and angiogenesis. At the top, vasculogenesis forms spontaneously new vessels from endothelial progenitor cells. At the bottom, angiogenesis sprouts new vessels from pre-existing ones. [2]
The blood vessels inner-wall interface or endothelium is involved in multiple and fundamental physiological processes including the diffusion of nutrients and oxygen, but also the protection from undesirable foreign elements. The last few years have pushed forward our understanding of human vascular growth during early and adult life, as well as its implications in pathologies. Several diseases take their origin in vasculature including cancer metastasis, migration thrombosis formation, atherosclerosis, immune response following inflammation, among others. To have a better understanding of these mechanisms, there is strong demand for in vitro physiological models of the vasculature.
Vascularisation in biomedical assays: shifting towards Organ-on-chip
Two-dimensional cells culture systems such as culture flasks have long been the gold standard method in life science research. However vasculature exhibits a complex 3D structure that cannot be reduced to conventional 2D models of the past. Moreover, experimental animal models have rapidly shown ethical concerns and the results are often not with what is observed in human beings. To address these limitations, engineered vasculature has rapidly emerged as a promising option for researchers. The last few years have witnessed an exponential growth of tissue engineering which offers great promises for human physiologically and pathologically relevant in vitro models. Emerging human-relevant organotypic models such as organoids and microfluidic organ-on-chips gradually replace the current conventional planar cell culture.
Organoids
Organoids are 3D cultured structures of self-organized, pluripotent stem cells, that are similar to actual human organs in various manners [3]. The potential of organoids is becoming widely considered in biomedical research, since they can provide closer model to the human organ physiology. Additionally the organoids can be used to accurately study the formation and progression of diseases. However in published organoid studies, there are few approaches to systematically produce robust vascularized organoids, limiting their use in vascular disease studies. Efforts have recently been made to implement vasculature in human organoids. However, most of these studies are static, meaning they do not experience any physiological flows or physical constraints, as can be observed in human organs.
Organ-on-Chip
Organ-on-chips are microfluidic platforms that enable the 3D culture of cells in dynamic conditions. Thanks to their size and confined environment, microfluidic devices can be used to mimic an organ structure and function, approximating human physiology. In these conditions, cells have shown to retain viability an differentiation level for increased period of times. More specifically, microvascular networks have recently been designed by combining microfluidic approaches and vascularization methods. These microfluidic-based models can implement biological and biophysical principles of the vascular system to better understand the blood-endothelium interface in both homeostatic and diseased conditions.
Studies carried out by Homan et al. on vascularization of organoid structures have suggested the crucial importance of integrating biophysical cues into our models [9]. This team implemented a flow-enhanced vascularization kidney organoid in vitro and reported that culturing kidney organoids within a microfluidic chips continuously perfused with laminar flow enhanced the expansion of endothelial progenitors and generated a vascular network. Furthermore, static cultures maintained the avascular and immature phenotype of kidney organoids, confirming the importance of physical forces and the potential of a microfluidic chip for vascular network development.
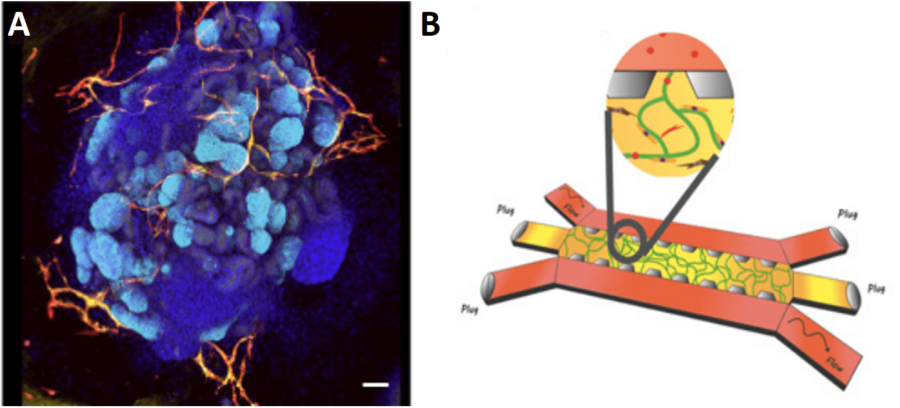
Implementing in vitro vascularisation in engineered models
As suggested above, microfluidics is valuable for the development of microfluidic vasculature-on-chip models and microtissues. Microfabrication methods have been extensively used to generate such complex interfaces. They indeed provide an unprecedented level of control over geometries (length, diameter, porosity…), in both hydrogels and polymers, guaranteeing precision down to the micron scale [6]. Various techniques are considered to build and pattern rapid perfusable vessel-like structures. They can be either classified as patterned networks, where the form and functions of the vasculature are artificially generated or as self-assembled, where the vasculature grows on its own inside of the microfluidic device. Here, we review these categories and provide insightful applications.
On Chip Vasculature Formation: Patterned networks
Biocompatible materials are first processed by additive micro-molding, requiring a pre-patterned template. This method is quite common and has been popularized by PDMS-based soft lithography. It is based on an “additive” approach where a flat structure is bonded with a patterned one forming the structure of the network. Polymers, as well as hydrogels, can be used in micro-molding methods, and although polymers present a stronger structure, hydrogels are highly porous and better mimic the structure of the in vivo extracellular matrix [7]. This method involves a straight-forward protocol, but ensuring correct and smooth bonding brings technical challenges, since a wrong alignment may cause leakage in the device.
For example, Zheng et al. engineered a microvascular network within a collagen matrix, to support angiogenesis and thrombosis assays [8]. After two weeks of endothelial cell culture, a functional vessel network embedded in a three-dimensional collagen scaffold was obtained (Figure 3). This relevant cell barrier demonstrated long-term stability, suitable for the study of vascular phenomena, such as thrombosis and angiogenesis.
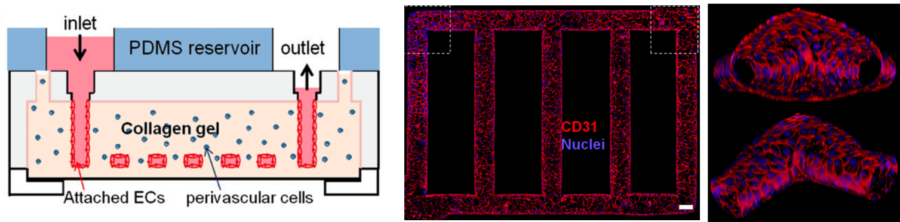
Figure 3 – Microfluidic vessel networks. On the left, the diagram of microfluidic collagen scaffolds. On the right, a horizontal section of the endothelialized microfluidic vessels, with a view at the corner [8].
The formation of patterned networks can also follow a “subtractive” approach consisting of the removal of the pre-patterned structure. Light-based methods are extensively used and rely on the photodegradation of the biomaterial. This method often requires the use of a template bulk that enables the selective irradiation of the biomaterial. The wavelength is tailored to the hydrogel properties and translated to the desired pattern [7].
Finally, sacrificial templating is the most common method of subtractive manufacturing. Sacrificial templating stands on the use of a sacrificial structure to generate perfusable microfluidic channels. Thin solid cylindrical rods, steel or even glass needles can serve as sacrificial structure (Figure 4). The resulting networks can be seeded with endothelial cells and cell culture media to grow a functional vessel. Generally, the sacrificial structure is introduced within a hydrogel, which can be easily tuned in terms of mechanical properties and composition. Once the hydrogel has polymerized, it is possible to remove the sacrificial rod to reveal an empty lumen. Endothelial cells (e.g., human umbilical vein endothelial cells, HUVECs) are then seeded and form a solid polarized barrier. Although sacrificial templating mimics the vascular cylindrical geometry and overcomes the limitations of solid polymeric channels, its design is restricted to uniaxial patterned channels with diameter above 100µm, whereas the smallest capillary vessels reach a few microns. To create more complex and interconnected networks, a specific mesh can be printed (e.g., 3D printed scaffolds) and encapsulated by the hydrogel, before being melted or dissolved. However, this technique demonstrates poor resolution [7].

Figure 4 – Micromolding : Removal of a sacrificial rod to generate a microfluidic channel [7]
On Chip Vasculature Formation: Self-assembled networks
Microfluidic designs using self-assembled networks are considered the closest to human microvasculature. They are particularly useful to study angiogenesis and vasculogenesis, and their associated pathologies. This method is based on the incorporation of 3D hydrogels inside microfluidic channels, acting as a suitable matrix for vascular growth [1]. Cells are seeded within the different channels according to a specific configuration – whether to mimic angiogenesis or vasculogenesis – and undergo spontaneous assembly into a network organization.
Kim et al. successfully generated and maintained a vasculogenesis primary vessel network over several days [9]. To do this, the authors used HUVECs as vascular precursors, seeding the cells inside a central channel, filled with a fibrin matrix supplemented with type I collagen. Fibroblasts (FB) were then used as stromal cells, to secrete pro-angiogenic growth factors into the lateral channels. After 5 days of culture, the authors observed an interconnected and perfusable vascular network with a functional lumen (Figure 5B). Moreover, angiogenic sprouting was induced within the microfluidic device when changing the seeding configuration (Figure 5F). This asymmetric growth and sprouting mechanism within the central matrix was initiated from the HUVECs toward the fibroblasts.
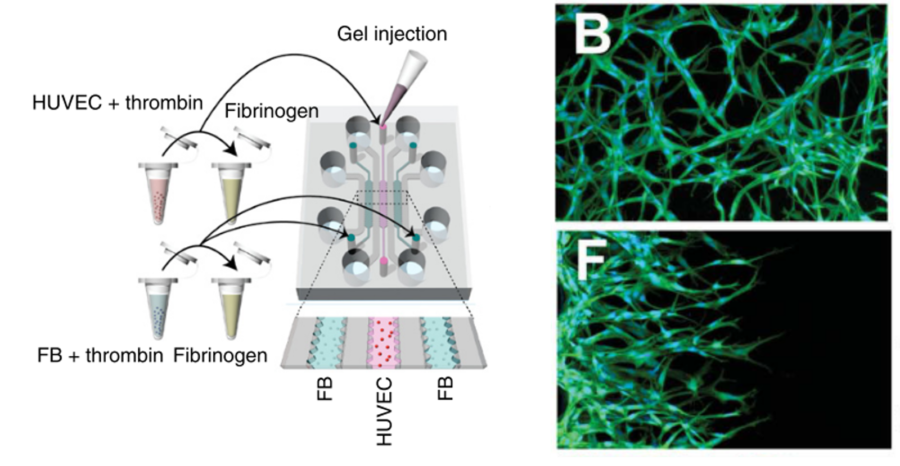
Figure 5 – Microfluidic chip design for vessels formation. On the left, schematic diagram of the microfluidic channels with a cell-seeding for vasculogenesis. The central channel is seeded in a 3D fibrin matrix whereas fibroblasts are embedded in a fibrin matrix as well and inserted in the external channels. On the right, HUVECs network formed by vasculogenesis (B) and angiogenesis (F) after 2 days of culture.
Engineered microvasculature can have many applications. Notably, Chen et al. developed a microfluidic platform for the generation of self-assembled networks to study the dissemination of tumor cells via circulation. As most metastases are found in the extravascular space, rather than the vascular lumen, in vitro 3D vascular models are of particular interest to shed light on the extravasation dynamics of tumor cells [10]. Figure 6 shows the dynamics of a tumor cell through an engineered vessel. This vasculature-on-chip device demonstrated the different steps of metastasis migration and further helped understand its underlying mechanisms.
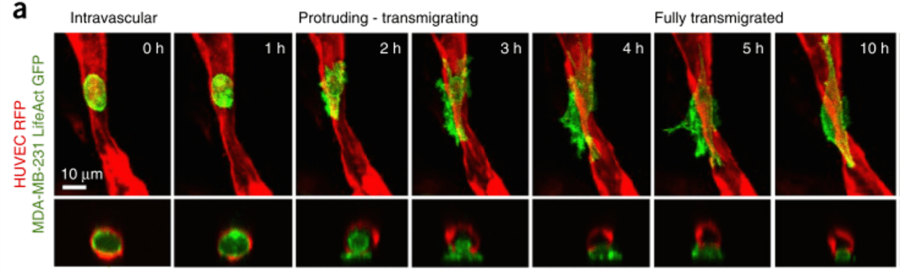
Figure 6 – Time-lapse sequence of a tumor cell extravasion. Endothelial cells form the microvasculature in red (HUVEC RFP), tumor cells in green (MDA-MB-231 LifeAct GFP) [10]
Future challenges for vasculature-on-chip
Vascularization for in vitro models has never been more important, whether to mimic vessel physiology or to study vascular disorders in different physiological conditions (hypoxia, fluid shear stress, chemical gradients…). Additionally, the blood vasculature is the main vector of communication between organs and is therefore essential to the development of the human-on-chip platform.
Biological parameters, such as cell phenotype, need to be considered when generating tissue-specific vascular networks. The use of hydrogels complexifies the engineered microenvironment and allows for the three-dimensional organization of relevant cell phenotypes, such as stromal cells, which were often not included in previous in vitro models and studies. Although the method of co-culture is well-known and is already implemented in organ-on-chip systems, it seems the next challenge will be the fabrication of devices capable of sustaining multiple cell types and physiologically relevant cell densities. The use of induced pluripotent stem cells (iPS cells) or human-derived primary cells also provide a valuable framework for disease understanding and patient-specific studies.
Finally, challenges remain in the development of scaffolds and biomaterials that more closely mimic human physiology. While most organ-on-chip devices are designed with PDMS (polydimethylsiloxane), next-generation organ-on-chips tend to be increasingly used for or in combination with vascularization-on-chip assays. In this respect, FlexdymTM is a soft thermoplastic elastomer developed by Eden Tech. This transparent biocompatible material allows for fast and easy fabrication of microfluidic devices within minutes. The fabrication of Flexdym chips is achieved through a user-friendly protocol using a hot-embossing machine (or hot press), providing a robust method to generate microfluidic chips for cell culture as seen in a recent publication by Salmon et al. in Figure 7 [11], [12].
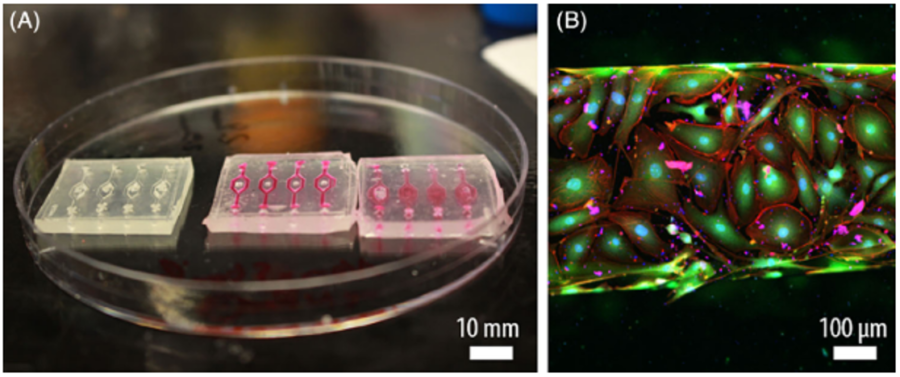
Figure 7 – Flexdym-based microfluidic chip. (A) Microchannels devices perfused with media. (B) Endothelial cells monolayer observed with a confocal microscope. [12]
Overall, there is still a long way to go for microfluidic vascular models to fully integrate biological needs in terms of drug and artificial vessels development. Microfluidics science is rapidly evolving and this dynamics is moving forward an even wider access to microfluidic technologies, fast and easy-to-handle processing methods for both Medtech industry and academics.
References
[1] Haase, K., & Roger, D. (2017). Advances in on-chip vascularization. 🔗
[2] Peak, C. W., Cross, L., Singh, A., & Gaharwar, A. K. (2016). Microscale Technologies for Engineering Complex Tissue Structures. Microscale Technologies for Cell Engineering, 3–25. 🔗
[3] Kim, J., Koo, B.-K., & Knoblich, J. A. (2020). Human organoids: model systems for human biology and medicine. Nature Reviews Molecular Cell Biology. 🔗
[4] Homan, K. A., Gupta, N., Kroll, K. T., Kolesky, D. B., Skylar-Scott, M., Miyoshi, T., Mau, D., Valerius, M. T., Ferrante, T., Bonventre, J. V., Lewis, J. A., & Morizane, R. (2019). Flow-enhanced vascularization and maturation of kidney organoids in vitro. Nature Methods, 16(3), 255–262. 🔗
[5] Jeon, J. S., Bersini, S., Gilardi, M., Dubini, G., Charest, J. L., Moretti, M., & Kamm, R. D. (2015). Human 3D vascularized organotypic microfluidic assays to study breast cancer cell extravasation. Proceedings of the National Academy of Sciences of the United States of America, 112(1), 214–219. 🔗
[6] Heintz, K. A., Bregenzer, M. E., Mantle, J. L., Lee, K. H., West, J. L., & Slater, J. H. (2016). Fabrication of 3D Biomimetic Microfluidic Networks in Hydrogels. 1–8. 🔗
[7] Tien, J., & Dance, Y. W. (2021). Microfluidic Biomaterials. Advanced Healthcare Materials, 10(4), 1–16. 🔗
[8] Zheng, Y., Chen, J., Craven, M., Choi, N. W., Totorica, S., Diaz-Santana, A., Kermani, P., Hempstead, B., Fischbach-Teschl, C., López, J. A., & Stroock, A. D. (2012). In vitro microvessels for the study of angiogenesis and thrombosis. Proceedings of the National Academy of Sciences of the United States of America, 109(24), 9342–9347. 🔗
[9] Kim, S., Lee, H., Chung, M., & Jeon, N. L. (2013). Lab on a Chip. 1489–1500. 🔗
[10] Chen, M. B., Whisler, J. A., Fröse, J., Yu, C., Shin, Y., & Kamm, R. D. (2017). On-chip human microvasculature assay for visualization and quantification of tumor cell extravasation dynamics. Nature Protocols, 12(5), 865–880. 🔗
[11] McMillan, A. H., Thomée, E. K., Dellaquila, A., Nassman, H., Segura, T., & Lesher-Pérez, S. C. (2020). Rapid fabrication of membrane-integrated thermoplastic elastomer microfluidic devices. Micromachines, 11(8), 1–19. 🔗
[12] Salmon, H., Rasouli, M. R., Distasio, N., & Tabrizian, M. (2021). Facile engineering and interfacing of styrenic block copolymers devices for low‐cost, multipurpose microfluidic applications. Engineering Reports, September 2020, 1–14. 🔗
Thomas Feaugas
Eden Medtech Ph.D. Candidate