Flexdym: Overcoming Challenges in Point of Care Diagnostics
Microfluidics is a research field focused on the microscopic manipulation of fluids that has origins in microelectronics. This technology has found diverse use in the development of novel biomedical devices, including diagnostic tests, biosensors, and drug development. Among various advantages, microfluidics allows the automation of experiments and increases user safety while decreasing space, time, and other costs – thanks to small reaction space and volume of reagents needed (Figure 1).
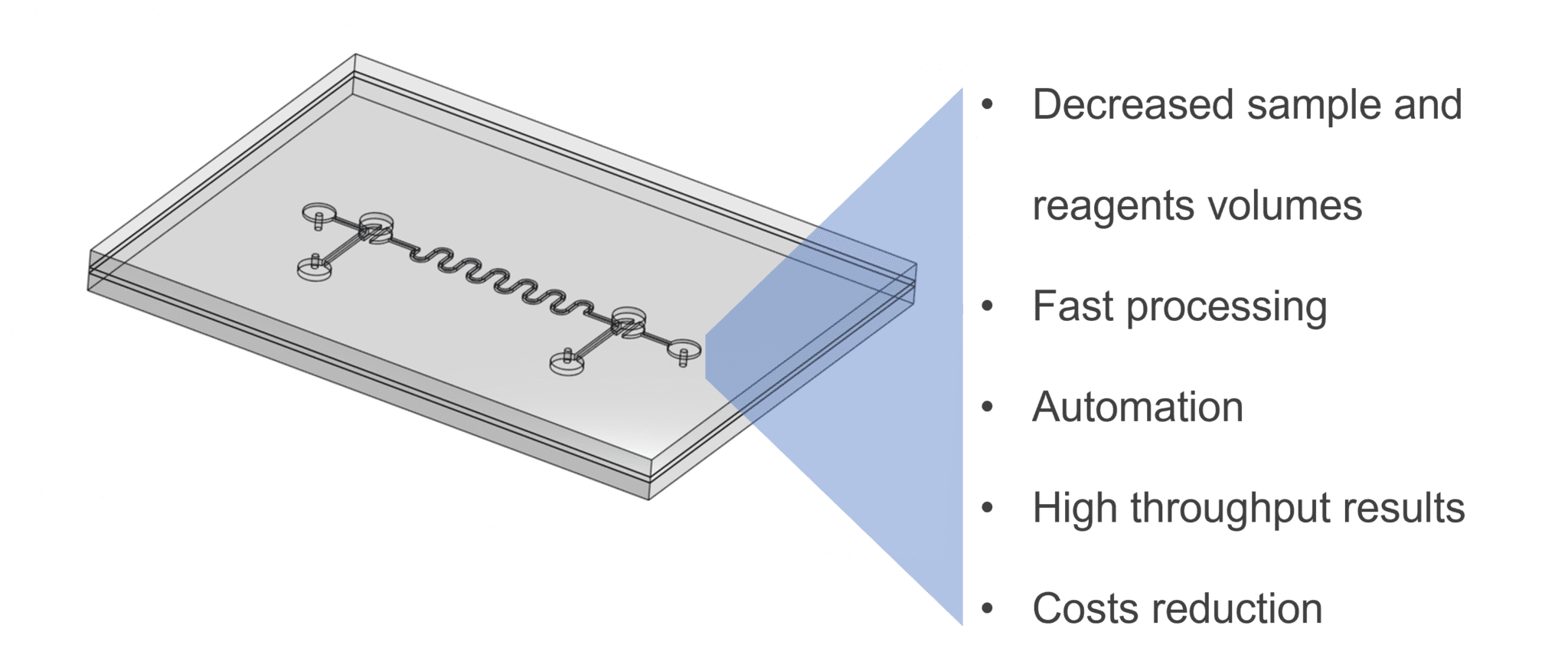
Point of care diagnostics refers to the analysis of medical conditions directly at the site where the patient is. Unlike clinical diagnostics, point of care diagnostics offers portability, rapid results, no required expert training, simple protocols, and cost-effective production.
There are multiple material options for the fabrication of microfluidic point of care devices. These can range from glass and silicon to polymers including polystyrene, COC, PMMA, and PDMS, with PDMS being the most popular in academic research. We will go through the characteristics and applications of these materials for the conception of microfluidic devices.
Finally, we present Flexdym™, a new alternative to PDMS, that is a thermoplastic elastomer easy to mold and assemble, that has ideal properties for the fabrication of devices for point of care diagnostics.
THE EMERGENCE OF MICROFLUIDICS
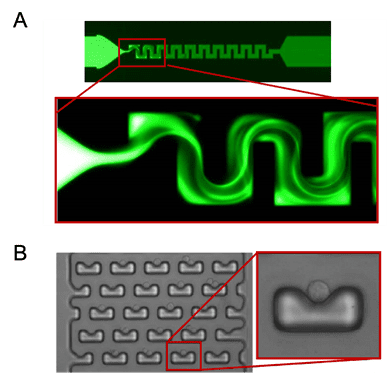
Figure 2: Example Geometries of microfluidic chips. Micromixer channel geometry allows mixing of fluids flowing through (A). Cell-trap structures responsible for capturing individual cells present in a sample (B)2,3.
Microfluidics is a technology based on the manipulation of fluids at the micrometric level. But how do microfluidic device’s function?
Microfluidics finds its roots in the microelectronics industry, – in fact, many materials and fabrication methods utilized in microfluidics derive from microelectronics. Throughout the years, the diversification of microfluidics towards potential applications in molecular analysis made it attractive for chemical and biological research4.
Initial microfluidics chips used silicon for their microfabrication; however, as the technology further developed for biological purposes, challenges such as material cost, and material properties (e.g. rigidity, transparency), led to the search for different materials for microfabrication of devices4,5.
Thanks to the benefits mentioned above the use of microfluidics has increased throughout the years. Microfluidics is present in various commercial products of the life science industry but also outside of it, some examples include:
Next-Generation Sequencing.
Illumina sequencing platforms based on sequencing-by-synthesis, use Flowcell devices. These are glass devices with multiple lanes containing billions of nano wells in which the clustered amplification of DNA fragments occurs. Sequencing is then accomplished from fluorescent detection of fluorescent-tagged nucleotides. In fact, this technology has allowed the increase of data output, a higher number of reads in faster run times, maximizing throughput results (Figure 3), its price can go up to $17,000 for the most performant machines. The NovaSeq 600 machine can perform up to 20 billion reads per run. Next-generation sequencing has tremendously reduced the cost and time of sequencing through the years, in 1990 the sequencing of the human genome took 13 years with a cost of 3 billion dollars. Today, to sequence a human genome takes only one hour for an average cost of 600 dollars.
Pharmacy pregnancy tests.
This well-known microfluidic technology is typically a Lateral Flow Assays (LFA) cassette. A liquid sample (e.g. urine) migrates by capillary action through a nitrocellulose membrane functionalized with two regions containing antibodies. These antibodies can recognize hCG, the hormone specifically produced by the body after fecundation. If the hormone is present in the sample, it is detected by the antibodies, and a colorimetric result appears in the form of two colored lines, meaning the test is positive (Figure 3).
Inkjet printers.
These machines contain microfluidic chips that generate ink droplets (10 to 100 μL) placed in specific locations of paper, leading to high-resolution images made from thousands of micrometric dots (Figure 3).
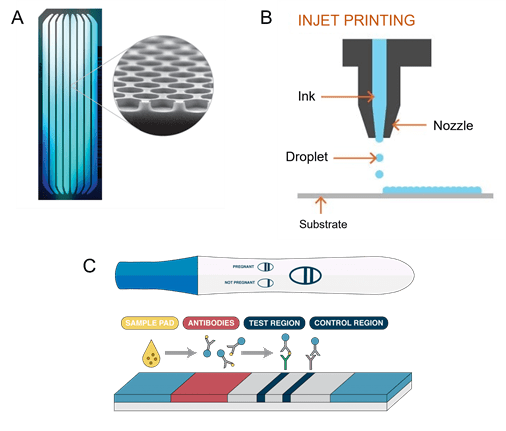
Figure 3: Devices incorporating microfluidics. Systems based on microfluidic processes. Illumina sequencing Flowcell device composed of multiple channels containing millions of nanowells (A); Pregnancy test based on capillary flow of liquid sample and antigen recognition by specific antibodies (B); Inkjet printers, deposits micrometric droplets on the substrate to create an image (C)6-8.
MICROFLUIDICS, A TOOL FOR POINT OF CARE DIAGNOSTICS
Traditional clinical diagnostic methods require a trained specialist, a range of laboratory equipment and the need to operate in a medical facility or hospital9. Sometimes the analysis of the samples can even take place in a separate laboratory, meaning that results take a couple of days. For instance, in the case of diagnostic PCR reactions, the required equipment includes reagents, pipettes, centrifuges, thermocyclers, and proper software for the analysis of results10,11.
Microfluidics devices, integrating multiple functions, are known as Laboratory-on-a-chip (LOC) devices9,10 (Figure 4). The purpose of these devices is to miniaturize and automate laboratory functions such as heating, mixing, or sorting, made possible thanks to specific on-chip designs such as micromixers, microvalves, micropumps, or microseparators, to list a few11 (Figure 2). By designing different geometries, the biological sample can be automatically processed within one device.
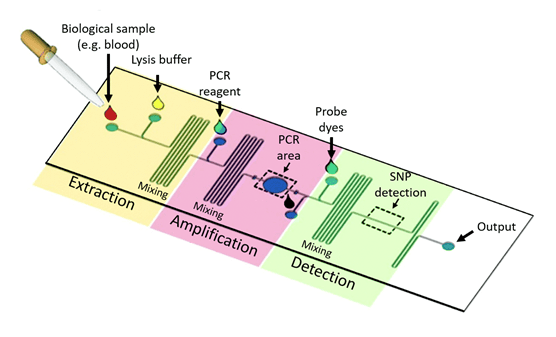
Figure 4: Laboratory on a chip. Schematic of a Lab-on-a-chip microfluidic device for PCR amplification. Different highlighted areas have a function: In the extraction region, the sample enters through the inlet of the device, and a lysis buffer enters through a second inlet, to extract the nucleic acid from blood cells in the sample. The serpentine channel serves as a mixer of the reagents. In the amplification region, PCR reagents (nucleotides, polymerase, primers, etc.) allow the amplification of DNA, taking place in the PCR area. In the SNP detection area, detection of amplified DNA occurs through fluorescent probes, and a compatible fluorescence captor12.
Point of care microfluidic devices are compatible with a range of fluid sample types (e.g. blood, urine, saliva, etc.), with only a few microliters needed to obtain fast results13. For instance, Watkins et al. have developed a microfluidic device for HIV diagnostics for fast counting of CD4+ and CD8+ T lymphocytes, which requires only 10 μL of whole blood and takes 20 minutes for determining a CD4/CD8 ratio14. In the case of certain infectious diseases scenarios such as Ebola, short detection times are critical. This is because Ebola disease causes severe hemorrhagic fever syndromes where patients die before antibody response15.
To rapidly detect Ebola virus, ReEBOV is a rapid diagnostic test that detects the presence of a viral antigen in blood samples. It is a Lateral Flow Immunoassay that is based on the same principle of previously stated pregnancy tests. In this situation, the antibodies present on the nitrocellulose membrane of the assay are specific for the recognition of VP40 matrix protein16.
The applications of point of care diagnostics devices are diverse, they can measure physiological parameters or detect pathologies, such as cancer, or diseases of different origins: genetic, epigenetic, metabolic, or infectious. The objective is to test a biological sample and detect molecules or agents that will give information about the health state of the patient. Common target analytes include nucleic acids, small molecules proteins, and cells17,18. Some well-known examples of point of care devices are:
Lateral Flow Tests
Cassettes for pregnancy diagnosis through the detection of hCG hormone present in urine (human chorionic gonadotropin)19.
Devices for HIV infection diagnosis through the detection of gp41 (glycoprotein 41) antigens20.
Devices for COVID-19 diagnosis through the detection of the viral RNA, or nucleocapsid (N) antigens17.
Commercially Available Devices
Accu-Chek Active by Roche, which monitors glucose in whole blood samples by using an electrical current which is interpreted17.
iSTAT-Systems by Abbott, is a portable device utilizing cartridges based on silicon with electronic sensors for the analysis of blood17.
We have contemplated the diversified potential of microfluidics. Let’s delve into the use of point of care and their principle in two specific contexts which are cancer biomarkers analysis, and SARS-CoV-2 detection the diagnosis of COVID-19.
Microfluidics for Cancer Diagnosis
In cancer diagnosis, tissue biopsies are the predominantly used method for the study of tumors, consisting of the extraction of a small fragment of an organ or tissue for analysis. This procedure can be quite invasive, surgically complicated, and sometimes life threatening for the patient.
Therefore, another alternatives for the molecular analysis of cancer is the liquid biopsy. Liquid biopsy procedures on the detection of circulating biomarkers in fluids such as urine, blood or saliva. Liquid biopsies have the advantage of being less invasive, highly sensitive, providing information from different locations of the body, and increasing the frequency of testing to monitor disease evolution17. Circulating biomarkers may have various origins, including from the apoptosis or necrosis of tumor cells, or from active release from tumor cells or healthy cells21–23. Biomarkers which have been successfully detected via microfluidic liquid biopsy methods include:
Circulating tumor cells (CTCs).
They present in the blood after metastatic release from the tumor site (Figure 5). Various microfluidics technologies have been developed for the detection of CTCs. For example Poudineh et al. developed a device capable of detecting less than 10 CTCs/mL of blood, indicative of metastasis in patients24.
Circulating Nucleic Acids.
They are released from cancer cells, such as cfDNA or miRNA. In a colorectal cancer study, Schwarzenbach et al. observed a correlation between shorter survival from patients with concentrations of cfDNA of 1000 ng/mL or higher25.
Proteins.
They can be present in the bloodstream in abnormal concentrations. For instance, Prostate-Specific Antigen (PSA) is a protein produced by the prostate, with normal blood concentration around 4 ng/mL. Higher concentrations (4-10 ng/mL) could be indicative of prostate cancer26.
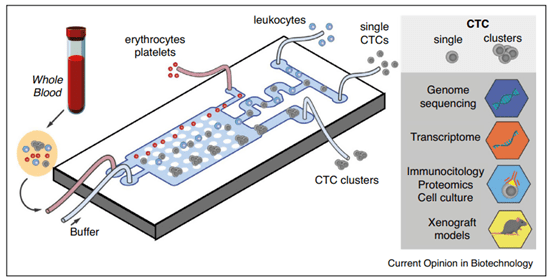
Figure 5: Microfluidics molecular assay for Cancer. Representation of a microfluidic device for diagnosis, able to separate and detect CTC or CTC clusters from a blood sample. The principle consists of depleting whole cell components onto different outlets and sorting single and clustered CTCs through lateral deterministic displacing methods. Single and cluster CTCs analyses are possible by different means such as DNA sequencing, proteomics, cell culture, etc21.
Microfluidics for COVID-19 Diagnosis
Microfluidic point of care diagnostics devices have also become a useful tool during the pandemic for the detection of the SARS-CoV-2 virus. One example of SARS-CoV-2 detection is in droplet microfluidics. Droplet microfluidics involves the distribution of single molecular or cellular targets into droplet compartments. Using a specific geometry, generation of water and oil micrometric emulsions permits the detection and quantification of targets. Inside each compartment the RT-PCR mixture is present, to perform a biochemical reaction and obtain rapid results (30 minutes)27. Alternative, point of care diagnostics can rely via SARS-CoV-2 antigen immunodetection assays built into disposable cassettes. These again, are lateral flow tests, which rely on flow of the sample through a membrane by capillary action. If the antigen is present, specific antibodies in the test region of the assay recognize it17,27.
MATERIALS FOR MICROFABRICATION OF DEVICES
One of the most important considerations when fabricating a microfluidic device is the material used. Microfluidic devices are very diverse, they are produced for specific applications, and there are countless designs that exist to accomplish different purposes. The material used depends on the function and goal of the device.
Microfabrication of devices have features molded or patterned on the surface of the material. These features can be a network of channels, chambers, pillars, wells, or other microstructures. The molded material is then assembled to a flat substrate (same or different material) in a bonding process, which closes the system28.
The first materials used in microfabrication were silicon and glass. This is because microfluidics and microfabrication processes have developed from microelectronics, which used silicon as the main support material thanks to its thermal and chemical stability, electrical conductance, easy scale-up for mass production, and standardized photolithography manufacturing process29. However, the development of alternative materials for easy and low-cost production, which provide other traits such as optical transparency, biocompatibility and elasticity has become important for biological applications. Polymers, such as thermoplastics and thermosets, have filled this void and provided further options for innovators working on the development and microfabrication of microfluidic devices. In the case of biomedical applications, there are key material properties researchers may require:
Optical transparency, for visualization of fluorescent dyes and tags in order to obtain readouts from a given experiment (e.g. measurements of SYBR green in DNA amplification, exited at 500 nm). Typical fluorophores wave lengths go from 450 to 700 nm.
Elasticity, allowing deformations, useful for the implementation of features such as quake valves.
Resistance to organic solvents, it can be used in microfluidic experiments (e.g. acids), with no damage to the device structure.
Surface properties, the Hydrophobic/hydrophilic properties of a material are important, possibility of surface treatments of the material (interesting for the functionalization with biomolecules on the surface of the channels).
Heat resistance, when the reaction must be activated at specific high temperatures (e.g. 95 ºC in PCR denaturation step).
Gas permeability, this is especially important for cell culture microfluidics to maintain adequate concentrations of oxygen for the cells.
Diversity in fabrication methods, multiple means for the fabrication of devices are possible, that can be accordingly suited for prototyping, mass production, rapid fabrication, etc.
Device fabrication involves three main steps: mold fabrication, device replication, and device assembly. In the fabrication of the master mold, available methods include subtraction or etching methods; additive or thin-film deposition methods; and photolithography. The latter is frequently used for its good resolution. Here, the technique consists on the curing of a photoresist (SU-8) layer with the desired patterns by projecting UV light through a photomask30.The second step is the replication of the design using methods such as soft lithography, etching, or hot embossing, among others. Device assembly differs according to the material but often involves using different chemical or thermal treatments. However, in point of care diagnostics devices are complex and require the integration of other components such as electrodes for sensing or reagent blisters, which makes bonding a challenge. Here we cover the most used polymers for microfluidics biotech applications:
Thermosets. These materials are irreversibly polymerized, leading to a solid end product, which cannot be re-melted, and therefore is non-transformable or recyclable. Thermosets result from the coupling of a resin with a crosslinking agent. The three-dimensional structure formed is stable and is thermally, mechanically and chemically resistant. The average Young Modulus is 7 to 35 GPa.
Thermoplastics. A thermoplastic is a material that can melt when heated above a certain temperature, but below that temperature it becomes hard again. Such materials will always retain their thermoplasticity. This feature makes thermoplastics potentially recyclable. This implies that the softened material is not thermally degraded and that the mechanical shear stresses introduced by a shaping process do not change the molecular structure of the material. Examples include polycarbonate (PC), PMMA, polystyrene (PS), or COC are thermoplastics. The average young modulus goes from 1 to 3 GPa.
Elastomers. An elastomer is a polymer with elastic properties, which can withstand strong deformations before breaking. The term rubber is a common synonym for elastomer. Polydimethylsiloxane (PDMS) is the most well-known example of an elastomer, used in microfluidic biological applications, with a Young Modulus of 1 MPa.
The development of PDMS for the microfabrication of devices in the early 2000s, represents a major breakthrough in the use of microfluidics for biological purposes. The PDMS microfabrication method of soft-lithography has become attractive thanks to its low-cost (compared to glass or silicon), easy to learn protocol, elastomeric properties, and straight-forward bonding process. Applications of PDMS include biochemical assays, chemical reactions, genomics, biomarkers detection, and cell culture studies.
PDMS was first developed by Whitesides and his team at Harvard, with a relatively simple protocol, consisting of soft lithography and oxygen plasma treatment, respectively31,4 (Figure 6). It is also considered to be relatively cost-effective for device production at small scales, and has attractive material properties such as optical transparency, softness, and gas permeability32. Finally, its elastomeric material properties provide key advantages in the conception of functional structures such as valves or actuation in devices.
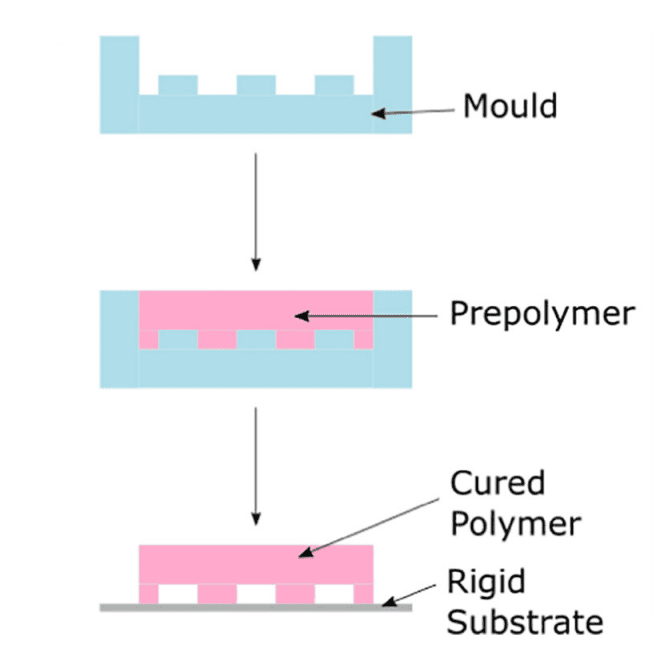
Figure 6: Soft lithography process. The polymer (PDMS) is put in contact with a negative patterned mold and cured for the obtention of a solid stamp with the positive pattern. Then the cured bonds to a rigid substrate for PDMS, a common substrate is glass4.
However, PDMS does present challenges in its microfabrication protocol and material properties – especially for industrial scale production. Like most polymers, PDMS is a hydrophobic material, which can gain hydrophilicity using a plasma treatment (e.g. oxygen or air), functionalizing the surface of the material with polar silanol groups (SiOH), for bonding with glass4. However, this effect is unstable because PDMS has a Tg (glass transition temperature) that is negative. This means that at room temperature, the polymeric chains within PDMS are in constant motion and those exposed to the plasma at the surface can easily relocate inside the material.
A major challenge in using PDMS for device fabrication is its incompatibility with mass production processes such as injection molding5. Although manufacturing of PDMS devices is possible by replica molding, the polymer is cast on a stamp, curing times are long (several hours) and the process lacks of automation, often leading to manual production. Thus, PDMS becomes a difficult candidate material to produce point of care devices.
Consequently, thermoplastics have become popular choices for commercial microfluidic devices, including point of care. They can provide transparency, biocompatibility, easy fabrication and scalability (e.g. through hot embossing or injection molding). Well-known and implemented thermoplastics include:
Cyclic Olefin Copolymer (COC). COC is a thermoplastic composed of ethylene and norbornene that has been used in the fabrication of lab-on-chip devices. COC is low-cost and exhibits good biocompatibility and transparency. It also presents high-temperature resistance, which makes it ideal for devices for thermo-dependent reactions such as PCR. However, some limitations are its hydrophobic surface properties, making it prone to spontaneous adhesion of cells or proteins – an issue when it comes to point of care devices measuring analytes33. Another limitation in POC device assembly is bonding that relies on chemical, thermal or adhesives. For instance, thermal bonding can cause partial melting of the material leading to channel deformation. In adhesive or chemical bonding, chemicals can react with biomarkers or reduce biocompatibility of the device.
Polystyrene (PS). PS is a thermoplastic which is rigid, transparent, and inert. Polystyrene is well-known for being biocompatible and widely used in cell culture, and it is used in the production of microplates and Petri dishes. Additional interests of using PS are its resistance to polar solvents such as alcohols, acids, and alkalis; and its low cost compared to other materials. PS is compatible with multiple industrial fabrication methods such as hot embossing, injection molding, or laser ablation34. However, the drawbacks concerning COC bonding, are also encountered in polystyrene device assembly.
Parylene is a thermoplastic polymer interesting for its use in flexible microfluidic devices. The biocompatibility and chemical inertness that characterizes this material allowed its implementation in the development of devices for biomedical applications. The flexibility of parylene makes it well adapted for tissue deformation. Some application examples of parylene devices are neural probes, microfluidic lens arrays, or an electrode coupled drug delivery system11, 35–37.
In the development of Lab-on-a-chip microfluidic devices, the device must often perform complex procedures therefore different microscale structures are integrated into the design. These include channels, pumps, reagent blisters, valves, micromixers, separators, chambers, electrodes, and membranes, among others10,11 (Figure 2). There is growing interest in the use of flexible materials as they can offer unique features. Opting for flexible material implies advantages such as mechanical resistance to higher flow rates without leakage; optimized mixing or valving processes; or similarity with biological entities, such as tissues and vessels. For example, valves allow the control of fluid flow and transport, such as the example illustrated in Figure 7, in a device made from PDMS38.
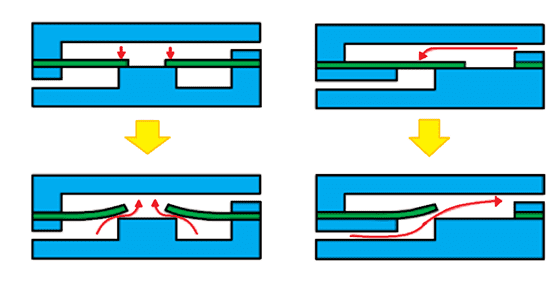
Figure 7: Representation of microvalves. Illustrated on the left, the mechanism of a diaphragm valve. On the right, the mechanism of a flap valve. In both instances, when the liquid enters from the top channel of the device, the flexible valve blocks passage into the bottom channel, and impedes flow. However, when the flow arrives from the bottom of the channel, the valves deform, allowing their opening and flow of the fluid11.
FLEXDYM™: A NEW MATERIAL FOR POINT OF CARE DIAGNOSTICS
Despite the availability of various materials for device fabrication, a large portion of biomedical devices use PDMS due to the perceived ease of replication with soft lithography and manageable small scale production costs. However, if the device proves to have commercial potential, product development may have to be re-initiated with a new material that is more easily scalable. So far, there has not been a material serving as a good alternative to PDMS, which can combine its best material properties with an easier production method and scale-up.
To help fill this existing gap between academia and industry, Eden Tech has developed and patented the Flexdym™, the first polymer created specifically for microfluidic biomedical applications.
Flexdym™ is a thermoplastic elastomer developed by Emmanuel Roy, co-founder and CEO of Eden Tech, who worked as a material scientist at the CNRC (Canadian National Research Council) on the development of point of care devices. In the following paragraphs, we will explain the many properties and benefits that make Flexdym™ an appealing material for the design of devices for point of care diagnostics.
Flexdym™ is a block copolymer, with dual physical properties. Its chemistry contains soft ethylene-butylene blocks and hard styrene blocks, providing flexibility and no treatment bonding, as well as compatibility with hot embossing microfabrication techniques used for thermoplastic molding39.
In terms of mechanical properties, the Flexdym™ polymer is flexible, exhibiting an elongation capacity of 720%, as well as a young modulus of 1.15 MPa, similar to PDMS (1MPa). However, it has a much higher tensile strength of 7.6 MPa, compared to 2.24 MPa for PDMS.
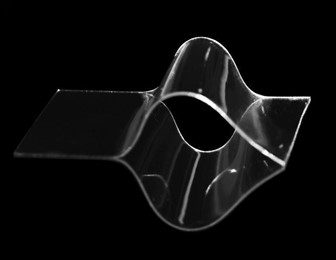
Figure 8: Flexdym™ sheet.
Flexdym™ is optically transparent, making it compatible with microfluidic assays requiring optical detection. It is characterized by high transmittance at UV and visible wavelengths appropriate to work with a large array of fluorophores and chromophores.
Moreover, the biocompatibility of the material makes it particularly adapted for the development of devices for point of care diagnostics. Flexdym™ material is certified USP Class VI and ISO 10993 parts 4,5,6,10, and 11.
“10993-4: Defines general requirements for evaluating the interactions of medical devices with blood.
10993-5: Defines test methods to assess the in vitro cytotoxicity of medical devices.
10993-6: Defines test methods for the assessment of the local effects after implantation of biomaterials intended for use in medical devices.
10993-10: Defines the procedure for the assessment of medical devices and their constituent materials with regard to their potential to produce irritation and skin sensitization.
10993-11: Defines requirements and gives guidance on procedures to be followed in the evaluation of the potential for medical device materials to cause adverse systemic reactions.”
In contrast to PDMS, Flexdym™ shows lower absorption of molecules. In a 2017 paper, Lachaux et al. exposed PDMS and Flexdym™ channels to a rhodamine solution for 24h and followed with a DI water wash. Fluorescence was measured on the walls of the channels for PDMS and Flexdym™. The intensity observed in PDMS was considerable compared to almost non detected fluorescence background for Flexdym39 (Figure 9). This supports the low sorption properties of Flexdym™ in comparison to PDMS. These are important considerations as absorbed molecules could affect experimental results.
Flexdym™ is resistant to acids, bases, alcohols (methanol & ethanol), and fluorinated oils. Additionally, gas permeability of the Flexdym™ for oxygen is 7.86 Barrer & for CO2 is 15.99 Barrer. This is roughly 30x lower gas permeability than PDMS, which is sufficient for cell culture experiments, one of Flexdym™ ’s top applications.
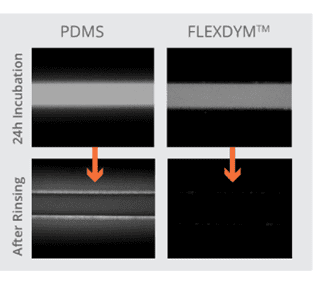
Figure 9: Rhodamine B adsorption. This figure shows fluorescence microscopy results for the remaining measured fluorescence of microfluidic channels after Rhodamine B incubation of 24 h followed by washing Left channel is made from PDMS and fluorescence is observed after rinsing. Right channel is made from Flexdym™, no fluorescence is observed after rinsing the Rhodamine B solution39.
Another asset of Flexdym™ is its reusable and recycling capacity. Flexdym™ can be washed and sterilized through gamma rays, autoclave, or ethylene oxide. And thanks to its thermoplastic properties, Flexdym™ devices can be heated and remolded for the fabrication of new devices.
The replication of devices with Flexdym™ is major advantage, as it is extremely fast, user-friendly, and low cost. It is done by hot embossing. In this replication method, parameters of temperature, pressure, and time are used to flow the material into features on a mold. It can take as little as 30 seconds to obtain the desired design on the Flexdym™, depending on the resolution and aspect ratio.
The chip assembly and bonding process is also simple and very straightforward. It is achieved through manual or automated lamination at room temperature overnight, or at 85°C for 1-2 hours, depending on the substrate material and its grade. Flexdym™ can be bonded to a variety of substrates such as PDMS, thermoplastics (i.e. COC, PC, PS, PI), glass or silicon, with bonding strengths ranging from 700 mbars to 2 bars (pressures tested so far).
Flexdym™ is also available in three different formats: starter packs, rolls, and pellets (Figure 10). The Flexdym™ starter pack includes 10 sheets (15 x 15 cm), which are ideal for initial testing of the material for small-scale production purposes. The Flexdym™ pellets are the most cost-effective format and best option for mass production, because they are compatible with industrial injection molding and 3D printing. Finally, the Flexdym™ rolls can either serve as a method to boost your production, as they are more cost-effective than the starter pack, or to move into device mass production, as they can be used for roll-to-roll embossing. As such, Flexdym™ is an ideal tool for academic research and initial industrial prototyping, but also compatible for large-scale mass production of commercial products – finally providing innovators with a material option that takes them from start to finish in technology development28.
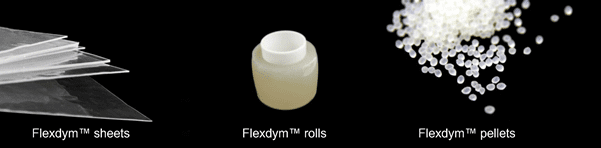
Figure 10: Flexdym™ formats. Available formats of Flexdym™ for academic and industrial microfabrication of devices for point of care diagnostics.
Conclusion
Microfluidics provides important benefits to the biomedical domain thanks to integrated Lab-on-a-chip devices that can serve various medical purposes such as, point of care diagnostics, and improve the treatment of patients. However, it is important to carefully consider the properties of the materials used for the fabrication of these devices to ensure that it is the best option for the device purpose.
Flexdym™ is a new revolutionary material created for easy microfabrication and with the user experience in mind. Its rapid and easy hot embossing replication protocol is suitable for academic research as well as industrial microfluidic biotechnologies, due to its compatibility with industrial production (i.e. injection molding, roll-to-roll embossing, 3D printing).
Finally, Flexdym™ is a cost-effective microfabrication technology for microfluidics, available on demand, and allowing innovators to save considerably in expenses compared to other materials. These savings occur at various levels including raw material costs, equipment costs, and the hours spent in the laboratory.
References
1. https://grabcad.com/library/pcr-microfluidic-chip-1.
2. Hejazian, M. Microfluidic mixing and jetting devices based on SU8 and glass for time-resolved molecular imaging experiments. in Microfluidics, BioMEMS, and Medical Microsystems XVII vol. 10875 59–65 (SPIE, 2019).
3. Carlo, D. D. Dynamic single cell culture array. Lab Chip 6, 1445–1449 (2006).
4. Convery, N. 30 years of microfluidics. Micro and Nano Engineering 2, 76–91 (2019).
5. Sackmann, E. The present and future role of microfluidics in biomedical research. Nature 507, 181–189 (2014).
6. https://emea.illumina.com/science/technology/next-generation-sequencing/sequencing-technology/patterned-flow-cells.html.
7. https://www.tt-99.top/products.aspx?cname=thermal+ink+jet&cid=6.
8. https://hybridoma.com/2020/08/24/antibodies-for-humanity/ (2020).
9. Azizipour, N. Evolution of Biochip Technology: A Review from Lab-on-a-Chip to Organ-on-a-Chip. Micromachines 11, 599 (2020).
10. Abgrall, P. Lab-on-chip technologies: making a microfluidic network and coupling it into a complete microsystem—a review. J. Micromech. Microeng. 17, R15–R49 (2007).
11. Fallahi, H. Flexible Microfluidics: Fundamentals, Recent Developments, and Applications. Micromachines (Basel) 10, 830 (2019).
12. Huang, C.-W. Efficient SNP Discovery by Combining Microarray and Lab-on-a-Chip Data for Animal Breeding and Selection. Microarrays 4, 570–595 (2015).
13. Srinivasan, V. An integrated digital microfluidic lab-on-a-chip for clinical diagnostics on human physiological fluids. Lab Chip 4, 310–315 (2004).
14. Watkins, N. N. Microfluidic CD4+ and CD8+ T lymphocyte counters for point-of-care HIV diagnostics using whole blood. Sci Transl Med 5, 214ra170 (2013).
15. Kaushik, A. Towards detection and diagnosis of Ebola virus disease at point-of-care. Biosens Bioelectron 75, 254–272 (2016).
16. Broadhurst, M. J. ReEBOV Antigen Rapid Test kit for point-of-care and laboratory-based testing for Ebola virus disease: a field validation study. Lancet 386, 867–874 (2015).
17. Sachdeva, S. Microfluidic Point-of-Care Testing: Commercial Landscape and Future Directions. Frontiers in Bioengineering and Biotechnology 8, 1537 (2021).
18. Vashist, S. K. Point-of-Care Diagnostics: Recent Advances and Trends. Biosensors (Basel) 7, 62 (2017).
19. Chard, T. REVIEW: Pregnancy tests: a review. Human Reproduction 7, 701–710 (1992).
20. Figueroa, C. Reliability of HIV rapid diagnostic tests for self-testing compared with testing by health-care workers: a systematic review and meta-analysis. The Lancet HIV 5, e277–e290 (2018).
21. Garcia-Cordero, J. L. Microfluidic systems for cancer diagnostics. Current Opinion in Biotechnology 65, 37–44 (2020).
22. Haber, D. A. Blood-based analyses of cancer: circulating tumor cells and circulating tumor DNA. Cancer Discov 4, 650–661 (2014).
23. Mader, S. Liquid Biopsy: Current Status and Future Perspectives. Oncol Res Treat 40, 404–408 (2017).
24. Poudineh, M. Profiling Functional and Biochemical Phenotypes of Circulating Tumor Cells Using a Two-Dimensional Sorting Device. Angew Chem Int Ed Engl 56, 163–168 (2017).
25. Schwarzenbach H. Detection and monitoring of cell-free DNA in blood of patients with colorectal cancer. Ann N Y Acad Sci. 2008 Aug;1137:190-6. doi: 10.1196/annals.
26. Kim, J. S. Prostate-Specific Antigen fluctuation: what does it mean in diagnosis of prostate cancer? Int Braz J Urol 41, 258–264 (2015).
27. Safiabadi Tali, S. H. Tools and Techniques for Severe Acute Respiratory Syndrome Coronavirus 2 (SARS-CoV-2)/COVID-19 Detection. Clin Microbiol Rev 34, e00228-20 (2021).
28. Nguyen, H.-T. Low-Cost, Accessible Fabrication Methods for Microfluidics Research in Low-Resource Settings. Micromachines (Basel) 9, E461 (2018).
29. Van Lintel, H. T. G. A piezoelectric micropump based on micromachining of silicon. Sensors and Actuators 15, 153–167 (1988).
30. McDonald, J. C. Fabrication of microfluidic systems in poly(dimethylsiloxane). Electrophoresis 21, 27–40 (2000).
31. Whitesides, G. M. The origins and the future of microfluidics. Nature 442, 368–373 (2006).
32. Regehr, K. J. Biological implications of polydimethylsiloxane-based microfluidic cell culture. Lab Chip 9, 2132–2139 (2009).
33. Jena, R. K. Micro fabrication of cyclic olefin copolymer (COC) based microfluidic devices. Microsyst Technol 18, 159–166 (2012).
34. Li, H. Fabrication of polystyrene microfluidic devices using a pulsed CO2 laser system. Microsyst Technol 18, 373–379 (2012).
35. Foley, C. P. Flexible microfluidic devices supported by biodegradable insertion scaffolds for convection-enhanced neural drug delivery. Biomed Microdevices 11, 915–924 (2009).
36. Pellinen, D. Multifunctional flexible parylene-based intracortical microelectrodes. Conf Proc IEEE Eng Med Biol Soc 2005, 5272–5275 (2005).
37. Seo, S. Parylene based thin-film microfluidic lens array fabricated by iCVD nano-adhesive bonding. Polymer 181, 121763 (2019).
38. Unger, M. A. Monolithic microfabricated valves and pumps by multilayer soft lithography. Science 288, 113–116 (2000).
39. Lachaux, J. Thermoplastic elastomer with advanced hydrophilization and bonding performances for rapid (30 s) and easy molding of microfluidic devices. Lab Chip 17, 2581–2594 (2017).
Sebastian Bermeo
M.Sc. Chemistry and Life Sciences